|
 |
Feature Story |
|
 |
Superconductivity and the physicist's MUSE
|
If you were an experienced physicist, the last place you would expect to find superconductivity is in iron. Iron is a ferromagnetic material, which means that it can be magnetized by an external magnetic field and remain magnetized even after the external field is removed. The strong interaction with magnetic field disturbs the electrical flow within the material resulting in electrical resistivity. This electrical resistivity, by definition, is the opposite of the superconductivity in which electrons experience zero resistance.
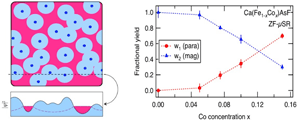
Left: Schematic view of superconducting phase (aqua-blue) in CaFe1-xCoxAsF in the sea of anti-ferromagnetism, and a cobalt atom (blue). Right: A plot of volumetric fraction of superconducting and magnetic media with respect to the percentage of cobalt.
|
 |
Thus, in February 2008, Prof. Hideo Hosono of the Tokyo Institute of Technology shocked the world with the discovery of a superconducting iron based compound: lanthanum oxygen fluorine iron arsenide, compound formula LaFeAsO1-xFx, or LFAO-F. Not only is this compound iron-based, its superconducting critical temperature--the temperature below which the material become superconducting state--is fairly high at 26 Kelvin (-247 degrees in Celsius). This surprising discovery brought attention and further experimentation from the worldwide superconducting research community. Other physicists, mainly from China, quickly learned that by replacing lanthanum with other rare-earth elements they could bring the critical temperature up to 55 Kelvin.
 |

Dr. Satoshi Takeshita of SPring-8, previously a post doc at KEK studying the superconducting phase in CaFe1-xCoxAsF.
|
This discovery presented the biggest challenge to the theoretical understanding of superconductivity since the discovery of the first high-temperature superconductors (cuprate compounds) in 1986. Before this point, physicists had not believed superconductivity could occur at 35 Kelvin. The most successful theory of superconductivity, known as BCS theory (after Bardeen, Cooper, and Schrieffer who were awarded the Nobel Prize for this work in 1972) prohibited critical temperatures above 30 Kelvin. Within a decade of the discovery of this first high-temperature superconductor, physicists had learned that they could increase the critical temperature of cuprate materials to 130 Kelvin. They did this by carefully replacing certain elements to produce a stronger electron-electron (hole-hole) coupling. Though it is thought that the strong electron-electron (hole-hole) coupling is a main ingredient of high-temperature superconductivity, the detailed mechanism is still not known.
The 2008 discovery of iron-based superconductors challenges the basic assumption that strong magnetism should disrupt superconductivity. Now, condensed-matter physicists at the Muon Science Establishment (MUSE) at the Japan Proton Accelerator Research Complex (J-PARC), are working to find out the mechanism of the iron-based superconducting materials.
How superconductors come to be
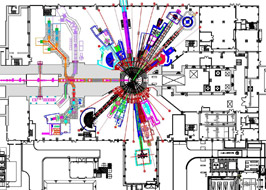
Schematic plan view of the neutron source (right) and muon beamlines.(left). Protons from the 3 GeV rapid cycling synchrotron (RCS) hit the muon target producing pions which decay into muons. Surface/decay muon channel (the blue lines on the left) is the only completed muon line so far. |
 |
"This was pure serendipity," says Prof. Ryosuke Kadono of KEK, the leader of the KEK-Muon Spin Rotation / Relaxation / Resonance (muSR) group. "There were no theories that predicted such an iron-based superconducting compound."
Like iron, Cobalt is also a ferromagnetic material. Hosono, Kadono, and their team members looked at a new high-temperature iron based compound (CaFe1-xCoxAsF) and found an intriguing feature of the superconductivity. When the team replaced some of the iron with cobalt, they found that superconducting spots started appearing in their sample. Given that superconductivity does not normally take homogeneity in material well, this was indeed surprising for these experts in the field. It is generally thought that electron pairs and hole pairs move around more easily in homogeneous medium.
At this time, the J-PARC MUSE facility was not yet completed, so they tested the sample at National Laboratory for Particle and Nuclear Physics (TRIUMF) in Canada. "We initially tested only two samples at cobalt ratios of 5% and 10%," says Dr. Satoshi Takeshita of SPring-8, then a post doc at KEK. Later the team tested some more samples to fill in the blanks at J-PARC MUSE. "I felt excitement when I saw the clear straight line on the graph."
The effect is curious. How would you picture the transition to superconducting state occurring inside a material? Does the entire sample of material gradually and evenly undergo phase transition? When physicists replace some of the iron atoms in CaFeAsF with cobalt atoms, a tiny area around each cobalt atom becomes superconducting. The size of superconducting 'islands' is 4-5 angstroms (one angstrom is 10-10 meter) in radius. At around 5% cobalt, these spots become numerous enough to be connected together, forming continuous paths for electrons to flow through the media without experiencing resistance. The total volume of the superconducting regions within the sample increases linearly with the amount of cobalt.
Muon Spin Rotation, Relaxation and Resonance
 |
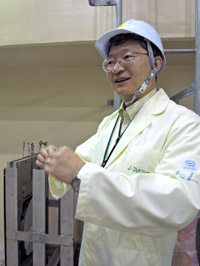
Prof. Yasuhiro Miyake of KEK, the leader of MUSE construction team, explains how he and his team designed the pillow seal (left corner).
|
By now, you may be wondering how the scientists are able to identify such microscopic superconducting structures. The enabling experimental technique is called Muon Spin Rotation / Relaxation / Resonance (muSR). Also called muon spin spectroscopy, muSR maps magnetic regions inside matter by means of muons shot into samples. A muon is an elementary particle that has a tiny-magnet like characteristic, called spin. Physicists can map a magnetic environment by looking at the way muon spin is affected by the magnetic fields produced by the surrounding structures, such as atoms and molecules. Because the superconducting regions exhibit special magnetic structure produced by super current, they are able to tell whether those tiny spots are superconducting.
At J-PARC MUSE, a team of MUSE scientists from KEK and Japan Atomic Energy Agency (JAEA) built a muon beamline to produce pulsed muon beams from the J-PARC's high intensity proton beams. The protons extracted from the rapid cycle synchrotron (3GeV) ring hit a graphite target and produce a bunch of pions. These pions then decay into muons in a six-meter solenoid. At this point, the muons' spins, or magnetic moments, are all perfectly aligned in the direction they are heading. Millions of these muons strike the surface of a sample, and slow down until they reach complete halt a few hundred micrometers into sample. This process is called implantation of muons.
These muons decay into positrons and other particles about 2.2 microseconds after production. When they do, they emit positrons preferentially in the direction of their spins, and from this physicists know the spin direction at the time of decay. Because muon decay time varies from muon to muon, they can plot out time evolution of muon spins as well. The KEK-muSR group at MUSE led by Kadono observed the spins of these decaying muons in their CaFeCoAsF samples, and used this information to reconstruct the distribution of magnetism within the material.
To the world's most intense muon beam
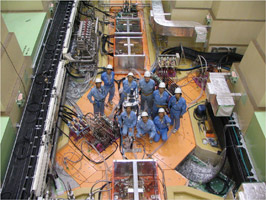
The MUSE construction team members at the muon target station. Pions and muons are extracted into four directions from this point.
|
 |
The world's first pulsed muon beam facility is the KEK's Meson Science Laboratory (MSL), which was built by a team of scientists at University of Tokyo in 1980. This was also where a team led by Steven Chu (then of University of California, Berkeley, now the head of the U.S. Department of Energy), conducted a successful precision experiment of dissociative excitation of thermal muonium, a hydrogen atom with the proton nucleus replaced with a positively charged muon, to test a theory called quantum electrodynamics.
This tradition of world leadership in muon science continues at MUSE, the only difference being that the positive muon beam will be two to three orders of magnitude more intense. Currently at 20 kilowatt, the power will be brought up to 100 kilowatt this fall. Eventually, the beam power will reach one megawatt. The first successful extraction of a muon beam occurred last September, and MUSE now attracts researchers from 14 universities and institutes.
 |
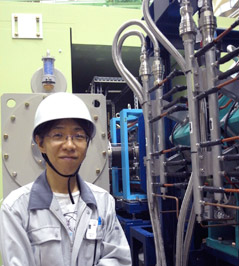
Dr. Wataru Higemoto of JAEA, in charge of the muon decay station (D1 area) above, explains how he designed the pulse slicer (aqua-blue).
|
One ingenious feature of muon beamline is the high time resolution of muon decay. For each muon implantation, scientists prefer narrower time window because then they will be able to tell more precise timing of muon decay. The pulse slicer installed near the end of the line was designed for this purpose. The slicer literally slices off the back end of a muon pulse to create a pulse with very narrow bandwidth of 30 nanoseconds by applying a steep electromagnetic pulse. "The high time resolution achieved from this is useful for the detailed observation of magnetic properties inside materials," says Dr. Wataru Higemoto of JAEA, who is in charge of one of the two muon experimental stations, D1 area, for the muon channel.
J-PARC MUSE is a remarkable product of precision engineering. "The challenge was to build everything to sub-millimeter precision with remote handling capability for radiological countermeasure," says Prof. Yasuhiro Miyake of KEK, the leader of the MUSE construction team. Such precision engineering paved the way for the timely application of muon to the newly discovered iron-based superconductor that blew physicists away. They are a team of just a dozen physicists and engineers. "Each one of us did the work of ten to complete the facility successfully and on schedule," Says Kadono. The muon channel is only phase one. There are still construction plans waiting for a new surface muon channel and a super omega muon channel.
At MUSE, muons will be produced in three different ways. Each method produces muons with a different energy. Higher energy muons are produced by the decay of those pions that pass through the graphite target. These are called decay muons, and their energies range from 10 MeV to 50 MeV. Moderate energy muons are called surface muons, and result from the decay of positive pions stopped at the graphite target. These particles have energies around 4 MeV. Then, there is the ultra-slow muon. Even the surface muons take 1 milimeter of matter to come to a complete halt. The super omega muon channel will deliver ultra-slow muons with energies in the range of 30 eV to a few tens of keV.
"In the next phase, we are interested in learning about what is happening just beneath the surface of materials," explains Kadono. He says that by studying the very near surface region of nanostructured composites, for example, scientists will possibly bring many novel discoveries to the nanotechnology. The micro-sized beams will also enable them to look into protein crystals and electron states in organic crystals. The science it probes does not stop there. From basic sciences such as particle physics, condensed matter physics, and chemistry, to applications such as muon catalyzed fusion, biophysics, noninvasive analysis, and beam technology, its applications are varied. "The proposed super omega channel has great potential to bring new innovations to the field of muon science," says Miyake. The world is waiting for such innovative machine to help explore the new science of matter.
|
|